BASIC ENVIRONMENTAL PHOTOBIOLOGY
Linda Chalker-Scott
Washington State University Puyallup Research and Extension Center
2606 W. Pioneer, Puyallup, WA 98371
lindacs@wsu.edu
The Informed Gardener Podcasts & The Garden Professor's Blog
What is Environmental Photobiology?
Many of the modules in
Photobiological Sciences Online focus on easily defined light phenomena: photosynthesis, vision, etc. Environmental photobiology has fuzzier boundaries, as it draws upon several fields of study, including photobiology, organismal physiology, and ecology. Thus, one might ask how a shade-loving plant is able to capture enough light to survive, how this ability is affected by increases in temperature or carbon dioxide, and how these interactions affect the ability of the plant to resist attack by insects.
Much of the focus of this module will be on plant systems. Plants are unable to move away from unfavorable environmental conditions, but they have evolved a number of mechanisms to cope with less than optimal levels of visible and ultraviolet (UV) radiation. Therefore, plants are especially in need of a system to sense whether the quantity and quality of available light is favorable for survival, growth, and reproduction.
What Happens When Plants are Exposed to Too Much Light?
Many algae and subcanopy terrestrial plants are well adapted to low light environments, and have extremely efficient light-harvesting systems. Under conditions where blue and red wavelengths have been selectively removed by other plants, shade plants are able to capture and use radiation in the yellow and green regions of the spectrum (Figure 1). [see module on
Modeling Leaf Optical Properties]
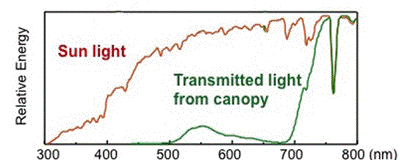
Figure 1. Comparison of full sunlight spectrum to that beneath a canopy of trees.
When low tides, deforestation, or other environmental shifts suddenly expose these species to higher levels of solar radiation, they accumulate light energy much faster than they can convert it to chemical energy. This overloading results in
photooxidation, a phenomenon that manifests itself through bleaching of leaf tissues. Depending on the sensitivity of the species and the severity of the exposure, this will either kill the plant or induce resistance strategies (Figure 2).
Figure 2. Sun intolerant plants (left) will photooxidize when exposed to excessive light (right).
Plants that are able to adapt to these new light conditions respond by growing leaves much different than those that were damaged or killed. These new leaves tend to be smaller and thicker, a strategy that decreases the surface area of the leaf, and thus decreases overall exposure. The upper leaf surface may be markedly different, with a thicker, waxier cuticle or a layer of hairs (trichomes), both which serve to increase surface reflectance, and thereby decrease radiation absorption. The color of the leaf may be altered as well: young leaves in particular often exhibit juvenile reddening, a phenomenon caused by the accumulation of
anthocyanins in the upper layers of the leaf. These red, blue or purple pigments can effectively decrease the amount of high-energy light (primarily blue, but some UV as well) that could damage the more sensitive photosynthetic tissue below (Chalker-Scott, 1999). Other related phenolic compounds are also important in this attenuation response.
What Happens When Plants Receive Too Little Light?
In contrast to shade-loving plants, sun plants are adapted to high levels of solar radiation, and possess protective leaf characteristics, such as those discussed above. If these plants receive less photosynthetically useful light through shading events, they will suffer starvation as their respiratory demands exceed their abilities to fix carbon. Sun plants enjoy unlimited solar energy, and are not efficient harvesters of light; unlike shade plants, they are unable to scavenge the less useful wavelengths. Such plants will develop chlorotic (yellow) leaves, and exhibit increased internode growth and phototropism (movement toward or away from light), as they attempt to escape low light conditions. [see module on
Basic Photomovement] Shade intolerant plants will not survive extended periods of low light conditions (Figure 3).
Figure 3. Sun plants will become chlorotic and etiolated when grown under shade conditions.
Some sun plants are shade tolerant, and their new growth exhibits characteristics similar to those found in shade plants. New leaves are larger and thinner, with thinner cuticles and reduced layer of hairs. This increases the light gathering efficiency of the plant by increasing surface area, and reducing reflecting compounds. There tend to be no filtering pigments present in the top layers of the leaves, but occasionally the lower leaf layers contain anthocyanins, which apparently reflect unabsorbed radiation back into the photosynthetic tissue.
How Does Light Induce Dormancy?
Those people who live in temperate climates where autumn is characterized by clear, sunny days and cold nights experience the fantastic color changes associated with deciduous trees entering dormancy (Figure 4).
Figure 4. Deciduous tree in the summer (left) and fall (right).
The function of this senescent process is to protect the tree's energy investments by scavenging, then removing, leaf tissue that would not survive the lethal freezing temperatures of winter. What surprises many people is that light is the environmental cue that trees use to initiate dormancy - not low temperature.
Dormancy that is imposed by, and relieved by, specific wavelengths of light is termed photodormancy. Photodormancy is associated with both winter dormancy and seed germination. The receptor responsible for this phenomenon is
phytochrome, a pigment that exists in one of two forms, depending on the wavelength of light it absorbs. What is important about phytochrome and its role in photodormancy is the relative ratio of the two forms of phytochrome (often termed "active" and "inactive" forms). Changes in this ratio can be observed daily, on a seasonal basis, and as a result of other introduced environmental factors. [see module on
Basic Photomorphogenesis]
At the summer solstice, temperate plants are exposed to the longest day of the year. Day length gradually shortens, and these changes are marked by changes in the ratio of the two forms of phytochrome. The ratio shift induces certain biochemical and physiological changes in the plant, which can be thought of as dormancy preparation. As fall begins, cold evening temperatures induce the second set of changes in plants; these changes are more obvious to us as they include leaf color changes. If it were not for the light-induced dormancy preparation, however, plants would not have time to respond to the cold temperatures, and would lose their leaves to frost damage before they were able to scavenge their energy reserves for storage in hardier tissues.
The same shifts in the ratio of phytochrome are also key in determining if and when seeds will germinate. Small seeds, in particular, tend to be photodormant. Consider what risks a seed faces if germination is initiated. While seeds themselves have a low water content, and can remain viable for long periods of time, actively growing seedlings are the most vulnerable life stage of plants. Germination is a race against time for plants, as they must find light and begin photosynthesis before their food reserves are depleted: the smaller the seed, the fewer reserves are available. Small seeded plants are best served from an adaptive viewpoint if they remain dormant until light conditions are favorable enough to allow photosynthesis.
How Does Light Induce Flowering?
If you live in a temperate region in any part of the world, the advent of spring is marked by the appearance of spring flowers - daffodils, tulips, crocus, forsythia. Other flowers appear throughout the season, each species rigidly adhering to the same flowering pattern year after year. This phenomenon was the subject of intense scrutiny early in the 20th century, when researchers defined the role of light in flower induction.
As noted, some species flower in the early spring, or in the fall, but never in the summer. Others flower during late spring and summer. Still others seem to flower all season long. Regardless of the flowering pattern exhibited by a particular species, those that are tied to specific seasons are controlled by phytochrome. The ratio of the two forms of phytochrome allows the plant to determine the day length and thereby the season. Those plants that bloom in the spring or fall are termed "short-day" plants, and are defined as plants generally requiring photoperiods of 10-12 hours (or less) to induce flowering. Conversely, "long-day" plants are defined as those requiring photoperiods of 12-14 hours (or more) to induce flowering. All others are categorized as "day-neutral" plants, as their flowering requirements are apparently not tied to photoperiod.
[
NOTE: This nomenclature, unfortunately, is not factually accurate, but has been retained in the interest of history. In actuality, phytochrome ratios are determined by the length of the dark period - not the light period. To be most accurate, we would call "short-day" plants "long-night" plants and "long-day" plants would be "short-night" plants. This historical nomenclature continues to distress botany students.]
How Does The Aquatic Light Environment Differ From Terrestrial Systems?
Like a tree canopy, water also selectively filters solar radiation. The first wavelengths absorbed are the longer, less energetic wavelengths in the red region of the spectrum; the blue wavelengths penetrate the farthest into the water (Figure 5).
Figure 5. Solar spectrum filtered through water.
Benthic plants, which live at the bottom of a body of water, and plankton are dependent on this filtered spectrum, and many of the macroalgae (e.g., seaweed) contain accessory pigments to absorb available radiation more efficiently. The red, brown, and golden algae are characterized by unique collections of accessory pigments, which do not occur in any of the vascular plant groups.
The relatively narrow photic zone of aquatic environments, which receives enough light to allow photosynthesis to occur, also poses a problem for planktonic plants and animals: phytoplankton (photosynthetic organisms) that sink too deep will eventually starve, as will zooplankton (non-photosynthetic organisms), which depend on this producer community. Plankton have evolved a number of morphological attributes that allow them to remain suspended in the water column or that actively propel them towards the sun (Figure 6). [see module on
Ultraviolet Effects on Phytoplankton]
Figure 6. Zooplankton (left) and phytoplankton (right) have structures to keep them afloat in the photic zone.
For zooplankton, migration towards the light is perilous: higher levels of solar UV can induce damage, and visual predators are more likely to see prey in well-lit areas. Thus, many zooplankters exhibit diurnal migratory behavior, rising to the surface when light levels are low and sinking as more radiation penetrates the water column.
How Do Other Environmental Factors Interact With Light?
The quantity and quality of light can affect how plants respond to other environmental factors. For instance:
Too little light limits photosynthesis, and reduces sugar accumulation, which in turn can prevent plants from surviving cold winter temperatures.
High intensity street lights can delay dormancy, and increase the occurrence of cold temperature damage.
High light levels can increase water loss, creating a drought stress on plants.
Plants that tolerate high light levels generally have increased tolerance to drought; the morphological and physiological changes associated with high light tolerance also reduce water loss.
Likewise, leaf adaptations to high light can reduce the amount of insect damage and disease.
|
Conclusions
The field of environmental photobiology continues to grow and develop as we become more aware of the interactions among individual organisms, their light environment, and other abiotic and biotic components of ecosystems. Because of their reliance upon solar energy for photosynthesis, plants are significantly influenced by differences in the amount and type of solar radiation present. Researchers in this field utilize discoveries made at the molecular, genetic, and cellular levels, and apply them to field situations. Of increased interest is the study of the response of organisms or communities to multiple environmental stresses; the effects of temperature, water, nutrients, insects, and disease upon organisms can affect, or be affected by, the light environment.
Suggested Reviews
Bjorn LO. (2008). Terrestrial daylight, pp. 123-130. In: LO Bjorn (Ed.) Photobiology: The Science of Life and Light, Second Edition. Springer Science + Business Media, NY, NY.
Chalker-Scott L. (1999). Environmental significance of anthocyanins in plant stress responses. Photochemistry and Photobiology 70:1-9.
Chalker-Scott L and A Cahill. (2001). Plant responses to natural and enhanced UV environments, pp. 231-250. In: TP Coohill and DP Valenzeno (eds.), Photobiology for the 21st Century, Valdenmar Publishing Co., Overland Park, KS.
McDonald MS. (2003). Photobiology of Higher Plants. John Wiley and Sons, West Sussex, England.
Roenneberg T and M Merrow. (2001). Seasonality and photoperiodism in fungi. Journal of Biological Rhythms 16(4): 403-414.
Smith RC and CD Mobley. (2008). Underwater light, pp. 131-138. In: LO Bjorn (Ed.) Photobiology: The Science of Life and Light, Second Edition. Springer Science + Business Media, NY, NY.
Spalding EP and KM Folta. (2005). Illuminating topics in plant photobiology. Plant, Cell and Environment 28(1): 39-53.
Weller JL and RE Kendrick. (2008). Photomorphogenesis and photoperiodism in plants, pp. 417-464. In: LO Bjorn (Ed.) Photobiology: The Science of Life and Light, Second Edition. Springer Science + Business Media, NY, NY.
03/24/10
05/13/12
[ TOP ]