ULTRAVIOLET EFFECTS on PHYTOPLANKTON
Donat-P. Häder
Neue Str. 9, 91096 Möhrendorf, Germany
donat@dphaeder.de
Aquatic ecosystems
cover about 70% of the surface of our globe. They produce an
amount of biomass that is equal to all the terrestrial ecosystems (e.g., forests and
grassland, agricultural areas and tundras and taigas) taken together.
The oceans represent the largest share in the aquatic ecosystems,
and all freshwater surfaces combined (lakes, rivers) account for only
0.5%. While macroalgae (kelp) are the major biomass producers in
coastal areas, phytoplankton are the predominant players in the open
sea.



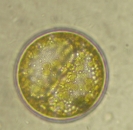
Left to right: Asterinella, Biddulphia, Ceratium fusca, Coscinodiscus
While usually being microscopically small and often unicellular,
these organisms grow at a fast pace, and often divide once a day. Sometimes they form dense algal blooms, which stain the water, Figure 1.
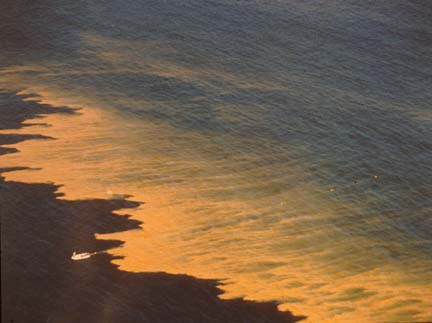
Figure 1. Phytoplankton bloom.
© Peter J.S. Franks, Scripps Institution of Oceanography.
Phytoplankton,
as the primary producers in the aquatic habitats, form the basis of
the intricate aquatic food web, Figure 2. They serve the primary consumers
(zooplankton such as larval fish and shrimp) as food, which in turn
are consumed by the secondary consumers (crustaceans, molluscs, fish,
birds and mammals including man). Thus, they ultimately provide a
significant share of the world’s animal protein for human consumption,
and in some parts of the world more than 50 % of the animal protein
comes from the sea. The third major component in aquatic ecosystems,
in addition to primary producers and consumers are the decomposers,
mainly bacteria, which break down living or dead biomass into inorganic
building blocks ready for reentry into the cycle.
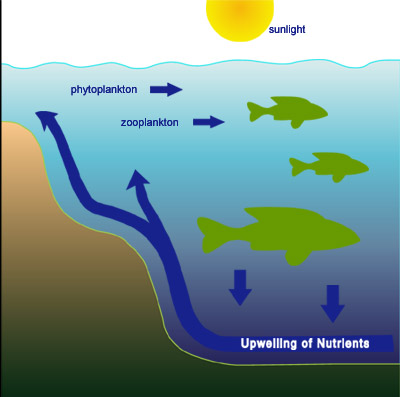
Figure 2. Marine Food Web. Phytoplankton are the primary producers.
They are consumed by zooplankton which are, in turn, consumed by progressively
larger aquatic organisms. The cycle completes as nutrients from deomposing
organisms circulate to supply nutritiion to phytoplankton.
Phytoplankton
organisms are a major sink for atmospheric carbon dioxide (CO
2) and
play a key role with respect to global warming, Figure 3.

Figure 3. Global
carbon fluxes and reservoir. The figure shows production (up arrows)
and consumption (down arrows) of carbon by (from left to right) aquatic
organisms, terrestrial organisms, industrial activity and forest fires.
The biological pump refers to carbon settling to the ocean bottom in
the form of decaying small particulate organic matter.
They absorb an
estimated 100,000,000,000 tons, or 100 Gt (1 Gt = 1 gigaton = 10
9
tons) of carbon from the total reservoir of 735 Gt in the atmosphere
(in the form of carbon dioxide). Most of this is recycled back into
the atmosphere when the organisms decay or, after being eaten, the
consumers decay. But part of the organic carbon sediments to the deep
sea floor where it is stored for hundreds of thousands of years, Figure
4.

Figure 4. CO2 transport in the sea. Two carbon cycles
are depicted that operate on vastly different time scales. Photosynthesis
and respiration cycles carbon into and out of the ocean on a time
scale measure in days. Dissolved organic carbon (DOC) and particulate
organic carbon (POC) accomplish the same cycling, but measured on
a time scale of centuries.
This sedimenting
particulate organic carbon (POC), called “oceanic snow”, can be collected and analyzed by traps installed at defined depths
(e.g. 1000 m) in the water column. This downward transport of carbon
out of the system is called the “biological pump” and
partially counterbalances the manmade input of carbon dioxide into
the atmosphere by fossil fuel combustion and tropical deforestation.
The phytoplankton is not equally distributed in the world’s
oceans. Highest concentrations are found in the subpolar regions and
in the upwelling areas of the continental shelves. This distribution
can best be seen from satellite images which show the chlorophyll
concentration in the water in false color images, Figure 5.
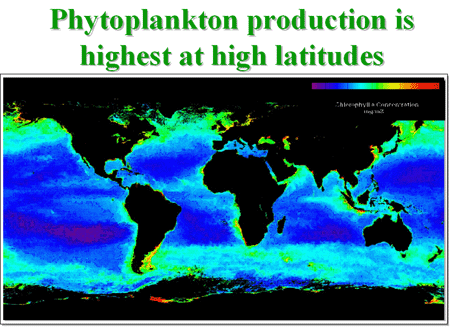
Figure
5. This false color image shows that the greatest phytoplankton densities
are near the poles and along continental shelves. The scale in the
upper reight corner indicates that blue is low density; green and
yellow are higher densities.
Being photosynthetic
organisms, phytoplankton are forced to move to, and to stay in, the
top layer of the water column illuminated by solar radiation, the
“photic zone”. The photic zone is not defined by its vertical
extension in meters but rather by the amount of available solar radiation.
The lower limit of the photic zone is the depth were the penetrating
light decreases to 0.1 % of the surface irradiation.
The availability of light depends on several factors. The spectrum
of light changes with depth as solar radiation penetrates to depth.
This is due to the absorption and scattering by the water itself as
well as dissolved and particulate organic and inorganic matter in
the water column. In coastal areas and freshwater ecosystems dissolved
organic carbon (DOC) plays a major role in attenuating the light penetration
and influencing the water color. DOC is a mixture of organic compounds
resulting from the breakdown of lignins, chlorophylls and other organic
material washed into the water by terrestrial runoff. DOC strongly
absorbs in the UV range of the spectrum. These organic compounds are
consumed by the bacterioplankton; however, bacteria find it difficult
to consume long-chained DOC. Solar UV breaks down these long molecules,
and the bacteria can consume the fragments faster. This increases
the transparency of the water and the penetration of solar UV to greater
depths. One feedback loop is that solar UV damages the bacteria, decreasing
their numbers.
Photomovement of Phytoplankton
Several phytoplankton
organisms use active mechanisms to move to and stay in the photic
zone: some have flagella or cilia and actively move in the water column
in search for optimal conditions for growth and reproduction. Others
use buoyancy for vertical migrations producing oil droplets or gas
vacuoles. Some of these movements follow a circadian rhythm with organisms
moving down at night and toward the surface before and during daytime.
Several members of the dinoflagellates have been found to swim 35
m up and down in the water column during their daily movements.
Phytoplankton
organisms use environmental signals to control their movements. Some
bacteria follow the magnetic field lines of the Earth (magnetotaxis).
Flagellates are known to detect thermal and chemical gradients of
oxygen and carbon dioxide. The most important clues are light and
gravity. In darkness actively motile phytoplankton organisms move
upward along the gravitational field of the Earth. This behaviour
is called negative gravitaxis (away from the center of gravity). This
orientation mechanism is truly remarkable. These unicellular organisms
perform the task of orienting in space, a task for which humans use
a complicated apparatus in their inner ear and a good amount of brain
power. The upward behaviour is supported by positive phototaxis (movement
toward the light) in the presence of low irradiance light. Many motile
phytoplankton organisms possess molecular photoreceptors and an internal
signal transduction mechanism to control their motor apparatus and
to orient with respect to the light direction.
In contrast to
the positive phototaxis just described, many phytoplankton cannot
tolerate the bright, unfiltered solar radiation at the ocean's surface
and are impaired especially by the high-energy, short-wavelength radiation
in the UV range of the spectrum. As a consequence, they use negative
phototaxis (movement away from a light source) or reversal of their
gravitaxis to move away from the water surface with its excessive
detrimental radiation. These antagonistic responses, which control
upward and downward orientation, result in an accumulation of the
organisms in a zone of intermediate and optimal light conditions,
Figure 6.
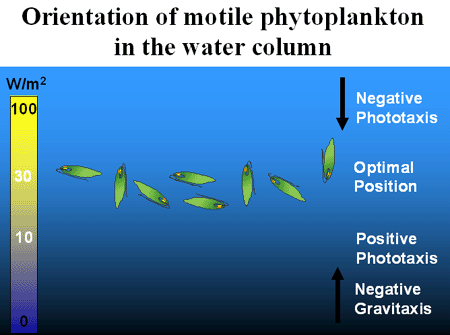
Figure
6. Phytoplankton near the ocean's surface can be harmed by
solar UV. Negative Phototaxis in this intense environment causes migration
to lower depths. If the phytoplankton are too deep insufficient illumination
causes a positive phototactic response, so the organisms move upward
to an optimal position. These
vertical migrations can be overruled by the action of wind and waves.
Of course, these
vertical migrations can be overruled by the action of wind and waves
in the top layer of the oceans, called the “mixing layer.”
The mixing layer extends from the surface downward to the level of
colder, unmixed water. The boundary between these layers is called
a thermocline. Even then the organisms bias their distribution in
the top layer of the ocean by active or passive oriented movements.
The distribution
of a phytoplankton population in the water column can be analyzed
by taking water samples from different depths and determining the
cell density. Figure 7 shows a typical situation during a calm day
near the island of Helgoland in the North Sea. The population mainly
consisted of dinoflagellates (
Ceratium) which moved upward
in the water forming a layer at the surface called a “neuston”
in the morning. Later in the day, around noon, the cells had moved
to a lower level. On the following day there was a strong wind. As
a consequence the phytoplankton organisms were equally distributed
within the mixing zone.

Figure 7. Density of the dinoflagellate Ceratium as
a function of depth below the surface at three times. Left panel -
morning of a calm day; middle panel - noon of the same day; right
panel - on a windy day. Early in the day, weak morning light is best
captured neaer the surface. At noon sufficient light penetrates more
deeply to depths where harmful UV cannot reach. Wind mixes all surface
layers preventing accumlulation at any depth.
[Key: red = green flagellates, green = Ceratium species, pink = total dinoflagellates]
Photodamage
and Photoprotection of Phytoplankton
Phytoplankton
organisms are in a dilemma: they are forced to move into the photic
zone due to their energetic requirements for solar radiation and on
the other hand they are affected by excessive solar radiation. Especially
short wavelength UV bleaches the photosynthetic pigments and breaks
down important other biomolecules including proteins and lipids. Under
the exposure of solar radiation chromophores form reactive oxygen
species (ROS) which affect structural and functional components of
the cell. Especially the genetic material of the cell, the DNA, is
a major target of solar radiation. Exposure to solar UV-B radiation
(280 – 315 nm) results in the formation of dimers of adjacent
thymine or cytosine bases in the DNA molecule, Figure 8.
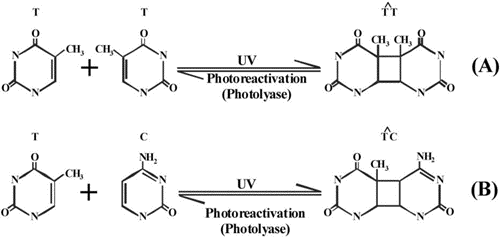
Figure 8. Thymine dimers.
If these chemical
modifications are not corrected they constitute mutations or result
in damage and death of the organisms. Fortunately, cells have developed
powerful mechanisms to identify and repair these UV-induced lesions.
One of the mechanisms involves an enzyme (photolyase) which uses the
energy of a UV-A/blue photon to break the dimer. Following the frequency
of dimer formation in a population of planktonic organisms shows a
drastic increase during daytime, especially if the organisms are located
close to the surface. In the late afternoon and evening hours these
lesions are repaired. The leftover lesions are then repaired overnight
using different molecular mechanisms (e.g. excision repair). A critical
situation arises when there is a carryover of lesions not repaired
before the next exposure, so that there is a build up of dimers in
the genetic material.
Other protective
mechanisms of phytoplankton rely on the absorption of solar UV by
a cellular “sunscreen”. Many phytoplankton (but also macroalgae)
produce one or more mycosporine-like amino acids (MAA) which absorb
in the UV range.
These
substances are located in the outer cytoplasmic layers and prevent
up to 7 out of 10 UV photons from reaching the central targets (e.g.
the DNA in the nucleus). The MAAs can be extracted from the cells,
Figure 9, and separated by HPLC, Figure 10.

Figure 9. Absorption
spectrum of a methanolic extract from G. dorsum exposed to
a solar lamp under a WG 305 filter for 24 h. Reprinted with permission
from JPP:B 60, 66.
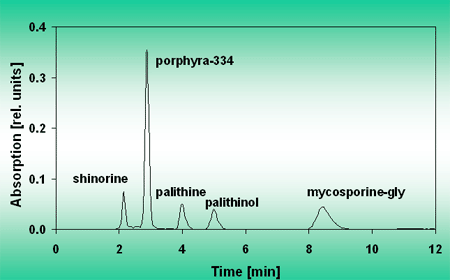
Figure 10. HPLC separation of MAAs.
The absorption
spectra of the separated MAAs show different absorption maxima spread
out over the UV-A region, Figure 11.
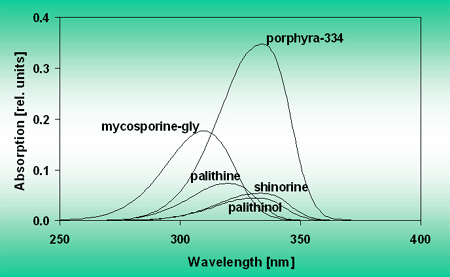
Figure 11. Library of various MAAs.
Some organisms
always have a certain level of MAAs. In others MAA synthesis is induced
by exposure to solar radiation. Recently measured action spectra show
that the biosynthesis is induced by UV radiation, Figure 12.
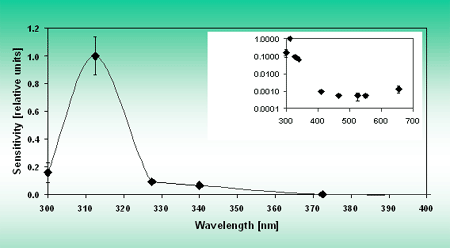
Figure 12. Polychromatic
action spectrum for MAA synthesis in G. dorsum. Inset shows
the action spectrum for the whole wavelength range on a logarithmic
scale. Reprinted with permission from JPP:B 60, 66.
Some organisms
are too small for even a high concentration of this sunscreen to be
effective. However, when analyzed on the population level protection
can be effective. For example, in microbial mats organisms in the top layer
absorb most of the detrimental UV radiation protecting the lower layers.
Active movement within the mat allows a fine tuned adjustment of the
light level that each individual receives.
Another target
of detrimental solar UV is the photosynthetic apparatus in the chloroplasts.
Solar energy is harvested by the photosynthetic pigments and transported
to the reaction centers where it is used to drive photolysis of water,
generation of energy and reducing substances required to build organic
molecules from water and carbon dioxide. Excess energy from the excited
chlorophylls can spill to molecular oxygen which then forms singlet
oxygen, a highly reactive molecular species which breaks down proteins,
pigments and other vital cellular biomolecules. In order to protect
the photosynthetic apparatus, the cells use certain carotenoids which
are located in close vicinity to the reactive centers and which absorb
the excess energy and relax it thermally before it can do damage to
the photosynthetic apparatus.
Another mechanism
for protection is “photoinhibition”: Excess solar radiation
kinks a central protein, referred to as D1, in the electron transport
chain from the reaction center chlorophyll
a to the primary
acceptor (phaeophytin). The damaged D1 protein is subsequently removed
by an enzyme called protease. This process reduces the electron transport
capacity of the photosynthetic apparatus and thus its vulnerability.
Photoinhibition can be measured in terms of photosynthetically produced
oxygen, Figure 13, or by the photosynthetic quantum yield.

Figure
13. O2 measurement in situ.
Pulse amplitude
modulation (PAM) fluorescence can be used to measure these processes
non-invasively by monitoring the fluorescence parameters.
After dark adaptation, phytoplankton show a high quantum yield of
up to 70% of all photons used for photosynthesis. When exposed to
excessive radiation this yield drops very fast to about 20%. When
returned to permissive light levels, there is a quick recovery to
the original levels. While most of this inhibition is due to visible
light (photosynthetic active radiation, PAR), a significant share
is due to UV radiation which accounts for much more than the energy
fraction in solar radiation, Figure 14.

Figure 14. Ulva rigida Photoinhibition and recovery. At
0 time, separate phytoplankton samples were exposed to PAR + UV A
+ UV B (grey bars), PAR + UV a (black bars) or PAR (white bars). The
quantum yield for photosynthesis is plotted as a function of time
after recovery.
Suggested Readings
Ferroni, L. Klisch, M. Pancaldi, S. and Häder, D.-P.: Complementary UV-absorption of mycosporine-like amino acids and scytonemin is responsible for the UV-insensitivity of photosynthesis in Nostoc flagelliforme. Mar. Drugs 8, 106-121 (2010)
Häder, D.-P.: Vertical migration and distribution of primary producers in aquatic ecosystems - The effects of enhanced solar UVB. Photochem. Photobiol. 65, 263-264 (1997).
Häder, D.-P. and Sinha, R.P.: Solar ultraviolet radiation-induced DNA damage in aquatic organisms: potential environmental impact. Mutation Research 571, 221-233 (2003).
Häder, D.-P., Hemmersbach, R. and Lebert, M.: Gravity and the Behavior of Unicellular Organisms. Developmental and Cell Biology Series, vol. 40. Cambridge Univ. Press 2005.
Häder, D.-P., Helbling, E.W., Williamson, C.E. and Worrest, R.C.: Effects of UV radiation on aquatic ecosystems and interactions with climate change. Photochem. Photobiol. Sci. 10, 242-260 (2011).
Häder, D.-P., Ntefidou, M., Iseki, M. and Watanabe, M.: Phototaxis photoreceptor in Euglena gracilis. In: Wada, M., Shimazaki, K. and Iino, M. (eds.): Light Sensing in Plants. pp. 223-229, Yamada Science Foundation and Springer-Verlag, Tokyo, (2005).
Helbling, E.W. and Zagarese, H. (eds.) UV effects in aquatic organisms and ecosystems. In Comprehensive Series in Photochemistry and Photobiology, vol. 1 (series eds. G. Jori and D.-P. Häder) RSC, London, 2003.
Klisch, M., Sinha, R.P., Helbling, E.W. and Häder, D.-P.: Induction of thymine dimers by solar radiation in natural freshwater phytoplankton assemblages in Patagonia, Argentina. Aquatic Sciences - Research Across Boundaries 67, 72-78 (2005).
Ntefidou, M., Iseki, M., Watanabe, M., Lebert, M. and Häder, D.-P.: Photoactivated adenylyl cyclase controls phototaxis in the flagellate Euglena gracilis. Plant Physiol. 133, 1517-1521 (2003).
Rastogi, R.P., Richa, Singh, S.P., Häder, D.-P. and Sinha, R.P.: Mycosporine-like amino acids profile and their activity under PAR and UVR in a hot-spring cyanobacterium Scytonema sp. HKAR-3. Australian Journal of Botany 58, 286–293 (2010).
Rastogi, R.P., Richa, Sinha, R.P., Singh, S.P. and Häder, D.-P.: Photoprotective compounds from marine organisms. J. Ind. Microbiol. Biotechnol. 37, 537–558, (2010).
Richter, P., Ntefidou, M., Streb, C., Lebert, M. and Häder, D.-P.: The role of reactive oxygen species (ROS) in signaling of light stress. Recent Res. Devel. Biochem. 4, 957-970 (2004).
Sinha, R.P., Helbling, E.W. and Häder, D.-P.: Effects of solar radiation on photosynthetic quantum yield of a cyanobacterium Nostoc sp. Trends Photochem. Photobiol. 10, 159-166 (2003).
Singh, S.P., Häder, D.-P. and Sinha, R.P.: Cyanobacteria and ultraviolet radiation (UVR) stress: Mitigation strategies. Aging Research Reviews. Online (2009).
Singh, S.P., Klisch, M., Sinha, R.P. and Häder, D.-P.: Sulfur deficiency changes mycosporine-like amino acid (MAA) composition of Anabaena variabilis PCC 7937: A possible role of sulfur in MAA bioconversion. Photochem. Photobiol. 86, 862-870 (2010).
03/04/08
09/09/11
[ TOP ]