Nature's Fantastical Palette: Color from Structure
Philip Ball
18 Hillcourt Road
East Dulwich
London SE22 0PE, UK
p.ball@btinternet.com
The changing hues of a peacock's splendid tail feathers have always captivated the curious mind (Figure 1). The seventeenth-century English scientist Robert Hooke called them 'fantastical' because the colors could be made to disappear by wetting the feathers (Hooke, 1665). Using the newly invented microscope, Hooke looked at peacock feathers and saw that they were covered with tiny ridges, which he figured might be the origin of the colors.
Figure 1. The shifting colors of the peacock's tail have had metaphorical interpretations for centuries.
Hooke was on the right track. The bright, often iridescent colors of bird plumage, insect cuticle and butterfly wings are 'structural'; produced not by light absorption by pigments, but light scattering from a regular array of objects just a few hundreds of nanometers (millionths of a millimeter) in size (Vukusic & Sambles, 2003; Vukusic, 2004; Wolpert, 2009). This scattering favors particular wavelengths depending on the size and spacing of the scatterers, and so it picks out specific colors from the full spectrum of sunlight. Because the precise hue may depend also on the viewing angle, structural colors are often iridescent, changing from blue to green or orange to yellow. And because they involve reflection rather than absorption, these colors can be startlingly brilliant. The Blue Morpho butterflies of South and Central America are visible from a quarter of a mile away, seeming almost to shine when sunlight penetrates the tropical forest canopy and bounces off their wings.
Structural colors are just one example of how living organisms manipulate and channel light using delicately arranged micro- and nanostructures. These biological designs offer inspiration to engineers seeking to control light in optical technologies, and could lead to more brilliant visual displays, new chemical sensors, and better storage, transmission and processing of information. To make effective use of such tricks, we need to understand how nature creates and deploys these tiny optical structures; indeed, we must learn a new language of color production and mixing.
Rather little is known about how many of these biological structures are put together, how they evolved, and how evolution has made creative use of the color and light effects they offer. But one thing is clear; nature doesn't have the sophisticated patterning technologies, such as drilling with electron beams, that microengineers can use to laboriously carve such structures from solid blocks. Ingenuity is used instead of finesse; these biological structures must make themselves from the component parts.
If we can master that art, we might develop new, cheap technologies to make such things as materials that change color or appearance, like the camouflage skins of some fish and squid, or fibres that guide and channel light with virtually no leakage, or chemically controlled light shutters. Here I look at some of nature's tricks for turning structure into color; and the ways they are being exploited in artificial materials and devices (Ball, 2012).
Layers
Although the ridges seen by Hooke on butterfly wing scales do scatter light, the bright colors of the reflected light generally come from invisible structures beneath the surface. In the natural world, they offer a robust way of generating color that is not hostage to the fate of delicate, light-sensitive organic pigments.
The colored scales and feathers of birds, fish and butterflies typically contain organized microscopic layers or rods of a dense light-scattering material embedded in a matrix of a different substance. Because the distance between the scatterers is roughly the same as the wavelengths of visible light, the stacks cause the wave phenomenon of diffraction, in which reflected waves interfere with one another. Depending on the angle of reflection, light rays of a certain wavelength interfere constructively when they bounce off successive layers in the stack, boosting the corresponding color in the reflected light (Vukusic and Sambles, 2003; Vukusic, 2004; Wolpert, 2009). It is much the same process that elicits the chromatic spectrum in light glancing off a tilted CD.
In butterfly wing scales the reflecting stacks are made of cuticle; a hard material containing the natural polymer chitin, separated by air-filled voids. In bird feathers, the stacks are platelets or rods of the dark pigment melanin; sometimes hollow, as in the Black Inca hummingbird,
Coeligena prunellei, embedded in keratin, the protein from which our hair and fingernails are made (Figure 2). Analogous diffraction gratings made from alternating ultrathin layers of two materials are widely used in optical technologies to select and reflect light of a single color. For example, mirrors made from multiple layers of semiconductors are used as reflectors and color filters in devices ranging from astronomical telescopes to solid-state lasers and spectrometers.
Figure 2. The iridescent blues and greens in the feathers of hummingbirds such as this Black Inca (left; part of blue iridescence highlighted with white box) are created by platelets of melanin pigment punctuated with air holes (right), which act as a photonic crystal to reflect light of a particular wavelength. K=keratin, A=air, M=melanin. (From Shawkey et al., 2009)
The male bird of paradise Lawes' parotia (
Parotia lawesii) has a particularly neat twist on this trick (Figure 3). The barbules (hair-like structures on the feather barbs) of its breast feathers contain layers of melanin spaced at a distance that creates bright orange-yellow reflection. But, as Stavenga and colleagues have recently discovered, each barbule has a V-shaped or boomerang cross-section, with sloping surfaces that also act as reflectors of blue light (Stavenga et al., 2011). Slight movements of the feathers during the bird's courtship ritual can switch the color abruptly between yellow-orange and blue-green; guaranteed to catch a female's eye. Stavenga suspects that technologists will want to use this trick for producing dramatic chromatic shifts. "I suspect the fashion or automobile industries will in due time make bent structures or flakes that will exploit these angular color changes", he says.
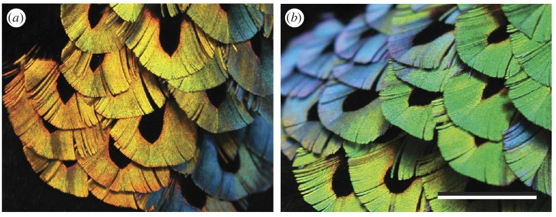
Figure 3. A striking color change in the feathers of the male Lawes' parotia, from yellow-orange (a) to blue-green (b), is caused by the presence of two mirror-like reflectors in the feather barbules (c): layers of melanin rods reflect yellow light, while the sloping faces of the boomerang-shaped barbule cross-section reflect blue at oblique angles. Scale bar in b: 1 cm. (From Stavenga et al., 2011)
Christmas Trees
The butterflies
Morpho didius and
Morpho rhetenor obtain their dazzling blue color not from simple multilayer's but from more complex nanostructures in the wing scales: arrays of ornate chitin 'Christmas Trees' that sprout at the surface (Vukusic & Sambles, 2003) (Figure 4). Each 'tree' presents a stack of disk-like layers to the incoming light, which acts as another kind of diffraction grating. These arrays may reflect up to 80 percent of the incident blue light. And because they are not flat, they can reflect a single color over a range of viewing angles, somewhat reducing the iridescence; organisms don't always want to change color or get dimmer when seen from different directions.
Figure 4. The butterfly Morpho didius (left) obtains its dazzling blue color from delicate 'Christmas Tree' light-scattering structures (right), made from chitin, that sprout within the wing scales. (Left, courtesy of Peter Vukusic. Right (micrograph) from Vukusic and Sambles, 2003.)
The precise color reflected depends on the refractive index contrast between the nanostructures and the surrounding medium. This is usually air, but as Robert Hooke observed, wetting such surfaces alters the refractive index contrast, and changes the color in a way that is closely linked to the wetting liquid's refractive index. For that reason, artificial Morpho-like structures carved into solids using microlithographic techniques are being developed by researchers at GE Global Research in New York, in collaboration with others at the State University of New York at Albany and butterfly-wing expert Pete Vukusic at the University of Exeter in England, as color-change chemical sensors that can identify a range of different liquids (Potyrailo, 2011). These might find applications for sensing emissions at power plants, monitoring of food safety, and testing of water purity.
Reflecting Bowls
The bright green color of the Emerald Swallowtail butterfly (
Papilio palinurus), found widely in southeast Asia, is not produced by green light at all. The wing scales are covered with a honeycomb array of tiny bowl-shaped depressions just a few micrometers across, lined with alternating layers of chitin cuticle and air which act as reflective mirrors. Light bouncing off the bottoms of the bowls is preferentially reflected in the yellow part of the spectrum. But from the sides it is reflected twice before bouncing back, and this selects blue. Our eyes can't resolve these yellow spots and blue rings, which merge to create the perception of green (Vukusic & Sambles, 2003).
Figure 5. The green of the Emerald Swallowtail butterfly (left) comes from the optical mixing of blue and yellow reflections from tiny bowl-like depressions in the wing scales (right). (Right figure, courtesy of Christopher Summers, Georgia Institute of Technology)
This way of making color has been copied by Summers and coworkers (Crne et al., 2011). To create the tiny bowls, they let water vapour condense as microscopic droplets, called breath figures, on the surface of a polymer dissolved in a volatile solvent. The solvent gradually evaporates to form a solid polymer film, while the water droplets pack together on the surface of the drying solution much like greengrocers' oranges and apples in crates, sinking into the setting film to imprint an array of holes. By pulling off the top part of the film, Summers and colleagues were left with a surface with hemispherical bowl-like dimples. They then used this structure as a template on which they deposited alternating thin layers of titania and alumina to make a multilayer reflector, like that lining the bowls of the butterfly wing scales (Figure 6).
Figure 6. An artificial micro-structured surface that mimics the green color of the Emerald Swallowtail. Scale bar: 5 µm. (Courtesy of Christopher Summers, Georgia Institute of Technology)
Because each reflection changes the polarization of the light, under crossed polarizing filters the yellow light bouncing back from a single reflection at the bowl centers disappears, while the twice-reflected blue-green light from the rims remains. This could offer a distinctive authentification mark on bank and credit cards. Apparently just a simple green reflective coating, such a material would in fact carry a hidden polarized signature in the reflected blue and yellow light that would be hard to counterfeit. But Summers' collaborator Mohan Srinivasarao admits that the main reason for seeking to replicate the butterfly's green color was that "it's beautiful in its own right".
Ordered Nanosponges
Scattering by regular arrays of microscopic objects can, for some arrangements, totally exclude light within a particular band of wavelengths, called the photonic band gap (Vukusic, 2004). These so-called photonic crystals occur naturally, for example, in opal, a biogenic form of silica in which the scatterers are tiny mineral spheres. Artificial photonic crystals can be used to confine light within narrow channels, creating waveguides that might be deployed to guide light around on silicon chips for optical information technology.
Nature has already got there first. Under the electron microscope, the wing scales the Emerald Patched Cattleheart Butterfly (
Parides sesostris) display zigzagging, herring-bone arrays: patches of an orderly sponge made from chitin with holes a hundred nanometers or so across. Each patch is a photonic crystal seen from a different alignment. Stavenga and Michielsen have found that these labyrinths in the wing-scales of
P. sesostris and some species of papilionid and lycaenid butterflies have a structure known to mathematicians as a gyroid (Michielsen & Stavenga, 2008). In
P. sesostris the structure has a photonic band gap that enables it to reflect light within the green part of the spectrum over a wide range of incident angles (Figure 7). Some weevils and other beetles also derive their iridescent color from three-dimensional photonic crystals made of chitin.
Figure 7. The wing scales of P. sesostris (top left, and close-up, top right) contain photonic crystals of chitin (bottom, middle and right) Scale bars: left, 100 µm; middle, 2 µm; right, 2 µm. (Bottom figure, from Saranathan et al., 2010)
Richard Prum and coworkers have figured out how these photonic crystals grow (Saranathan et al., 2010). The molecules in the soft membranes that template the deposition of chitin during wing-scale growth become spontaneously organized into the 'crystalline sponge'. Biological membranes are made up of long, tadpole-like molecules called lipids, which have a water-soluble head and an oily tail. To shield the tails from water, they cluster side by side into sheets with the heads pointing outwards; the sheets then sit back to back in bilayer membranes. Pores in these membrane induce curvature, partly exposing the lipid tails and therefore incurring a cost in energy. For this reason, the pores in effect repel one another, and this can force them to become arranged in a regular way, an equal distance apart. Periodic membrane structures have been found in the cells of many different organisms, from bacteria to rats (Hyde et al., 1997).
In
P. sesostris wing-scale progenitor cells, the outer 'plasma membrane' and the folded membrane of the inner compartments called the endoplasmic reticulum, where lipids and other molecules are made, come together to form a so-called double-gyroid structure (Figure 8, left), in which two interweaving sets of channels divide up space into three networks that interpenetrate, but are isolated from one another. One of these is then filled with chitin, which hardens into a robust form while the cell dies and the rest of the material is degraded, leaving behind the single gyroid phase (Saranathan et al., 2010).
It has been suggested that these natural nanostructures might be used as the templates for making artificial ones, for example, by filling the empty space around the chitin with a polymer or an inorganic solid, and then dissolving away the chitin (Saranathan et al., 2010). But it is also possible to mimic the structures from scratch. For instance, artificial bilayer membranes made from lipid-like molecules called surfactants will also form orderly sponges, and so will so-called block copolymers, in which the chain-like molecules consist of two stretches with different chemical composition (Hyde et al., 1997). Ulrich Wiesner and coworkers (Stefik et al., 2012) have mixed liquid block copolymers with nanoparticles of niobium and titanium oxide, and let the polymers form into gyroid and other ordered 'nanosponge' structures that usher the nanoparticles into the same arrays. When this composite is heated, the polymer is burnt away while the mineral nanoparticles coalesce into continuous networks (Figure 8, center).
These porous solids could find a wide range of uses. Thin porous films of titanium dioxide nanoparticles coated in light-absorbing dyes are already used in low-cost solar cells. These orderly gyroid networks can offer improvements, partly because the solid material through which light-excited electrons are harvested is continuously connected rather than relying on random electrical contacts between nanoparticles. And the researchers have calculated that double-gyroid nanosponges made from metals such as silver or aluminum, which might similarly be assembled from nanoparticles guided by block copolymers, could have the weird property of a negative refractive index, meaning that they would bend light 'the wrong way' (Hur et al., 2011). Such materials could be used to make so-called superlenses for optical microscopes that can image objects smaller than the wavelength of light; something that isn't possible with conventional lenses.
Inspired by the butterfly structures, Mark Turner and colleagues (Turner et al., 2011) have used laser beams to 'write' these intricate three-dimensional photonic crystals directly into a commercial light-polymerizable 'photoresist' material (Figure 8, right). Being somewhat 'scaled-up' versions of the natural nanostructures, these had photonic band gaps in the infrared part of the spectrum. Current telecommunications operates mostly at infrared wavelengths, and these structures could find uses there; some, for example, have a corkscrew lattice that make them respond differently to circularly polarized light with a left- or right-handed twist.
Figure 8. The gyroid phase (left), and structures mimicking the 'butterfly gyroid': (middle) a network of titania organized by self-assembly of a block copolymer, and (right) a larger-scale lattice made by setting a light-sensitive polymer with laser beams (scale bar: 10 µm). (Left figure, courtesy of Matthias Weber, Indiana University. Middle figure, from Stefik et al., 2012. Right figure, from Turner et al., 2011)
Photonic Crystal Fibers
The spines of some marine polychaete worms, such as Aphrodita (the sea mouse) and Pherusa, are tubular structures containing hexagonally packed hollow cylindrical channels a few hundred nanometers across and made from chitin. These arrays act as two-dimensional photonic crystals that reflect light strongly in the long-wavelength part of the spectrum, which gives the Aphrodite spine a deep, iridescent red color (Figure 9) (Parker et al., 2001; Trzeciak & Vukusic, 2009).
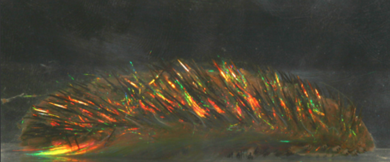
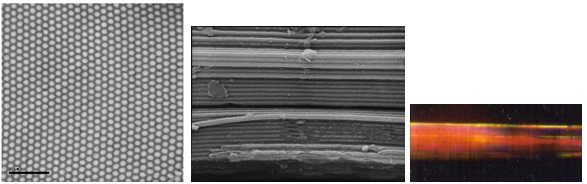
Figure 9. The tiny spines of polychaete worms such as the sea mouse (Polychaeta: Aphroditidae; top left) are natural photonic crystals. Seen close up in cross section, they consist of regularly packed hollow channels with walls of chitin. Middle left: cross-section from Pherusa (scale bar: 2 µm); center: side view of channels from Aphrodita; right: the red color of light passing through a spine of Aphrodita. Artificial photonic fibres like this can easily be made by heating and drawing out bundles of glass capillaries (bottom). They can confine light within the 'solid' channels even around tight bends. (Note the solid 'defect' in the central channel.) (Top, middle center and middle right, courtesy of Andrew Parker, University of Oxford. Middle left, from Trzeciak & Vukusic, 2009. Bottom, from Russell, 2003)
It is not clear if the optical properties of the polychaete spines have any biological function. But there are certainly uses for such light-manipulating fibres in optical technology. For example, Philip Russell and collaborators (Russell, 2003) have made them by stacking glass capillaries into hexagonally packed bundles and drawing them out under heat into narrow fibers laced through with holes. If 'defects' are introduced into the array of tubular channels, either by including a wider capillary or a solid rod in the bundle, light can pass along the defect while being excluded from the photonic crystal, creating an optical fiber with a cladding that is essentially impermeable to light of wavelengths within the band gap. Photonic crystal fibers like this can guide light around tighter bends than is usually possible with conventional fibers, where the light is confined less reliably by internal reflection at the fibre surface. As a result, these fibers would work better for guiding light in tightly confined spaces, such as on optical microchips. And because photonic crystal fibers are in general less 'leaky' than conventional ones, they could be replace them in optical telecommunications networks, requiring less power, and obviating the need for amplifiers to boost signals sent over long distances.
Disordered Nanosponges
The splendid blue and green plumage of many birds, while also being physical rather than pigmented colors, lacks the iridescence of the hummingbird or the peacock. Instead, they have the same color viewed from any angle. They scatter light from sponge-like keratin nanostructures; but because these structures are disordered, the scattering is diffuse, like the blue of the sky, rather than mirror-like and iridescent (Dufresne et al., 2009).
In the blue-and-yellow macaw,
Ara ararauna, (Figure 10), and the black-capped kingfisher Halcyon pileata, the empty spaces in the keratin matrix of the feather barbs form tortuous channels about 100 nm wide. A similar random network of filaments in the cuticle of the Cyphochilus beetle gives it a dazzlingly bright white shell. In some other birds, such as the blue-crowned manakin,
Lepidothrix coronata, the air holes are instead little spherical bubbles connected by tiny cavities.
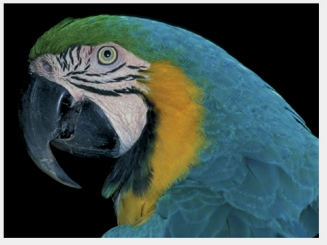
Figure 10. The blue feathers of the blue-and-yellow macaw contain sponge-like labyrinths of air and keratin (bottom left), which scatter blue light strongly in all directions. Some other feathers derive similar colors from spherical 'bubble-like' air holes in the keratin matrix (bottom right). Scale bars: 500 nm. (Bottom figure, from Dufresne et al., 2009)
It is believed that both of these structures are formed as keratin separates out spontaneously from the fluid cytoplasm of feather-forming cells, like oil from water (Dufresne et al., 2009). In liquid mixtures, such as solidifying molten metal alloys or polymers, such phase separation creates different structures in different conditions. If the mixture is intrinsically unstable, the components separate into disorderly, interwoven channels in a process called spinodal decomposition. But if the mixture is metastable (provisionally stable), like water supersaturated with dissolved gas, then the separating phase will form discrete blobs or bubbles that grow from very tiny 'seeds' or nuclei. Prum thinks that either of these processes may happen as bird feathers develop, and that birds have evolved a way of controlling the rate of keratin phase separation so that they can arrest the nanostructure at a certain size. Once the cells have died and dried, this size determines the wavelength of scattered light, and thus the feather's color.
This kind of diffuse light-scattering has been used for centuries as a way of making colors in technology. In milk, microscopic droplets of fat with a wide range of sizes cause scattering of all visible wavelengths, and give the liquid its opaque whiteness. Michael Faraday discovered in the nineteenth century that light scattering from nanoscale particles of gold suspended in water can create a deep reddish-purple color with a precise hue that depends on the size of the particles. Glassmakers had been using alchemical recipes to precipitate nanoscale gold particles in molten silica to make ruby glass ever since ancient times.
Today, engineers are looking at how these random networks and particle arrays can give rise to strongly colored and high-opacity materials. Pete Vukusic and colleagues (Hallam et al., 2009) have mimicked the cuticle of Cyphochilus beetles in random porous networks made from interconnected filaments of the minerals calcium carbonate and titanium dioxide mixed with a polymer and oil liquid binders and left to dry. Guided by the size and density of filaments in the beetle shell, they were able to make thin coatings with brilliant whiteness. Meanwhile Prum, his colleague Eric Dufresne and their coworkers at Yale University (Forster et al., 2010) have mimicked the disordered sponges of bird feathers by creating films of randomly packed microscopic polymer beads, which have blue-green colors (Figure 11).
Figure 11. This thin film of randomly arrayed polymer microspheres mimics the keratin matrix in the blue feathers of the blue-crowned manakin. (From Forster et al., 2010)
Color Change
One of the most enviable optical tricks in nature is to produce reversible color changes. The reflective, protean colors in the skins of squid such as the Loligidinae family are produced by a protein called reflectin, arranged into plate-like stacks in cells called iridophores, which again act as color-selective reflectors (Figure 12). The color changes are thought to be involved in both camouflage and communication between squid for mating and displays of aggression.
Figure 12. Stacked plates of the reflectin protein (left) in iridophore cells (center) create tunable reflective colors in squid (right). (Center figure, courtesy of Daniel Morse, University of California at Santa Barbara)
Daniel Morse and colleagues have recently figured out how the color changes of iridophores are achieved (Tao et al., 2010). The reflectin proteins crumple up into nanoparticles, which pack together into dense arrays that make up the flat layers. These layers are sandwiched between deep folds of the cell membrane. The color change can be triggered by neurotransmitter lipid molecules called acetylcholine, which activate a biochemical process that fixes electrically charged phosphate chemical groups onto the reflectin protein. These groups largely neutralize the proteins' intrinsic charge and allow them to pack more closely together, increasing the reflectivity of the layers. At the same time, this compaction squeezes water from between the protein particles and out of the cell, and enables the reflectin layers to sit closer together.
Morse and colleagues (Holt et al., 2010) think that it should be possible to copy some of these tricks in optical devices, perhaps even using reflectins themselves. They have inserted the gene encoding a reflectin protein from the long-finned squid
Loligo pealeii into
Escherichia coli bacteria. When expressed, the protein spontaneously collapses into nanoparticles (Tao et al., 2010). The size of these particles can be tuned by controlling the interactions between charged groups on the proteins using salt. Held between stacks of permeable membranes, these materials might therefore swell and contract, altering the reflected wavelengths, in response to chemical triggers. Morse and colleagues have also taken inspiration from reflectins to develop a light switch based on a wholly synthetic light-sensitive polymer. They use an electric field both to change the refractive index of the polymer and to pull salt into the polymer film to swell it. As with iridophores, this combination of effects alters the material's response to light dramatically, switching it from transparent to opaque; all without moving parts or high-tech manufacturing methods. The team are currently working with Raytheon Vision Systems, an optics company in Goleta, California, to use this system in fast shutters for infrared cameras.
The Art and Science of Natural Color Mixing
Many of the optical effects found in nature are not purely due to structural colors, but arise from their combination with absorbing pigments (Shawkey et al., 2009). In squid, a thin pigment layer above the reflective layer acts as a filter that can modify the appearance, for example, making it mottled; reflective and absorbing to different degrees in different places. In bird feathers, the physical colors resulting from melanin nanostructures embedded in a keratin protein matrix can be tuned by light-absorbing filters of pigments, such as carotenoids, which absorb red and yellow light. The characteristic green plumage of parrots seems to be produced by laying a yellow pigment over a blue reflective layer of melanin and keratin (Figure 13). And the purple wing tips of Purple Tip butterflies come from red pigments beneath a blue iridescent surface.
Figure 13. Green is a characteristic color of parrots, but their plumage contains no green pigment, nor is it purely a structural color. Rather, it results from 'structural blue' overlaid with a filter of yellow pigment.
Chameleons display perhaps the most advanced mastery of these mixing tricks. Their spectacular color changes are produced by three separate systems for modifying the reflected light, stacked one atop the other. The first layer consists of cells containing red and yellow light-absorbing pigment particles, the location of which within the cell determines the color intensity. Below these are iridophores like those of squid, from which blue and white light may be selectively reflected by crystalline layers of the molecule guanine (also a component of DNA). Finally there is a layer of cells containing the dark pigment melanin, which act like the colored 'ground' layers of Old Master paintings to modify the reflection of light that penetrates through the first two layers. This combination of reflection and absorption enables the chameleon to adapt its skin color across a wide, albeit species-specific, range to signal warning, for mating displays, and for camouflage (Forbes, 2009).
How pigments alter and adjust the reflected light in such cases is still imperfectly understood. One problem is that the combinations are so diverse; more than 20 different arrangements of melanin, keratin and air have been identified in the plumage of birds. Moreover, melanin is itself a light absorber, creating colors ranging from yellow to black. The bright white markings on the blue wings of the
Morpho cypris butterfly are produced by simply removing the melanin from reflective multilayer structures; the mirrors remain, but the pigments do not.
In such ways, evolution has made creative use of the limited range of materials at its disposal to generate a riot of profuse coloration and markings. A better understanding of how this is achieved could give painters and visual artists access to entirely new ways of making colors based on iridescent and pearlescent pigments, whose use has so far been largely restricted to less sophisticated applications in the automobile and cosmetic industries (Schenk & Parker, 2011).
Painter Franziska Schenk has been exploring the mixing of structural and pigmented color during her stay as artist-in-residence in the Department of Biosciences at the University of Birmingham in the UK (Schenk, 2009). With iridescent particles, says Schenk, "the established methods of easel painting no longer apply. Their conversion to painting requires something truly innovative."
Schenk used iridescent particles to reproduce the starting blue of the Morpho wing in a series of paintings that change color when lit or viewed from different angles (Figure 14). The background color on which the particles are placed is central to the effect. On white, the light not reflected from the blue particles passes through and bounces off the base. This means that when not seen face-on, the blue quickly fades and is replaced by a muted yellow. But on a black background, all non-blue light is absorbed, and the blue is more pure and intense.
Figure 14. Painting of a Morpho butterfly wing by Franziska Schenk, using blue pearlescent pigments. The color changes depending on the angle of illumination, as well as on the nature of the background color. (Courtesy of Franziska Schenk)
Although the brilliance of these colors doesn't approach that of butterfly wings, it takes advantage of recent improvements in synthetic pearlescent particles. The first of these were made by coating mica flakes with multilayers of metal oxides to generate the diffraction grating. But because the mica surfaces were not perfectly smooth and the grain sizes varied, there was always a range in the precise colors and intensities of the particles. Schenk has used pigments in which the mica substrate is replaced by a transparent borosilicate glass, which is smoother and gives a purer hue. She believes that "iridescent technology is destined to introduce a previously unimaginable level of intensity and depth, thus adding beauty, luster and a dynamic dimension to art". Schenk's Studies of Cuttlefish (Figure 15) is a painting that uses iridescent flakes mixed with beads and wax.
Figure 15. "Studies of Cuttlefish" by Franziska Schenk, using iridescent flakes mixed with beads and wax. (Courtesy of Franziska Schenk)
Another series of cuttlefish, "Mantle of Many Colours" (Figure 16), was made with iridescent paint that differs in appearance depending on the conditions and angle of lighting, which results in a compelling chameleon effect that traditional paints simply cannot create. The colors change from greens to purples as the viewing angle shifts. "Still images, together with any attempt to verbally describe the effect, are pretty limiting", Schenk admits; you have to see these things in the flesh to appreciate their full impact.
Figure 16. "Mantle of Many Colours" by Franziska Schenk, which uses iridescent paint, as seen from different angles. (Courtesy of Franziska Schenk)
Conclusion
"Every day you play with the light of the universe", wrote the Chilean poet Pablo Neruda, but he had no idea how literally true this would become. Our technologies for transmitting, manipulating and displaying information, whether for work or play, depend increasingly on our ability to control light; to harness and transform color. Some of nature's most stunning sights depend on such a facility too, and often they show us that beauty can be inextricably linked to utility. We are impressed by plumage, by markings and animal displays, that are specifically designed by evolution to make such an impression. And nature has found ways to make this chromatic exuberance robust, changeable, responsive, and cheap and reliable to manufacture. In shaping color without the chemical contingency of pigments, there seems to be little we can dream up that nature has not already anticipated, exploiting its capacity to fashion intricate fabrics and structures on the tiniest scales. We can only learn, and admire.
References
Note: The current article is an extended version of Ball P (2012), "Nature's color tricks", Sci. Am. 306(5), 74-79.
Crne M, Sharma V, Blair J, Park J O, Summers C J & Srinivasaro M (2011), "Biomimicry of optical microsctructures of Papilio palinurus", Europhys. Lett. 93, 14001.
Dufresne E R, Noh H, Saranathan V, Mochrie S G J, Cao H & Prum R O (2009), "Self-assembly of amorphous biophotonic nanostructures by phase separation", Soft Matter 5, 1792-1795.
Forbes P (2009), Dazzled and Deceived: Mimicry and Camouflage. Yale University Press, New Haven.
Forster J D, Noh H, Liew S F, Saranathan V, Schrenk C F, Yang L, Park J-G, Prum R O, Mochrie S G J, O'Hern C S, Cao H & Dufresne E R (2010), "Biomimetic isotropic nanostructures for structural coloration", Adv. Mater. 22, 2939-2944.
Hallam B T, Hiorns A G & Vukusic P (2009), "Developing optical efficiency through optimized coating structure: biomimetic inspiration from white beetles", Appl. Opt. 48, 3243-3249.
Holt A L, Wehner J G A, Hampp A & Morse D E (2010), "Plastic transmissive infrared electrochromic devices", Macromol. Chem. Phys. 211, 1701-1707.
Hooke R (1665), Micrographia. Reprinted by BiblioBazaar, p. 294. Charleston, South Carolina, 2007.
Hur K, Francescato Y, Giannini V, Maier S A, Hennig R G & Wiesner U (2011), "Three-dimensionally isotropic negative refractive index materials from block copolymer self-assembled chiral gyroid networks", Angew. Chem. Int. Ed. 50, 11985-11989.
Hyde S, Blum Z, Landh T, Lidin S, Ninham B W, Andersson S & Larsson K (1997), The Language of Shape. Elsevier, Amsterdam.
Michielsen K and Stavenga D G (2008), "Gyroid cuticular structures in butterfly wing scales: biological photonic crystals", J. R. Soc. Interface 5, 85-94.
Parker A R, McPhedran R C, McKenzie D R, Botten L C & Nicorovici N-A P (2001), "Aphrodite's iridescence", Nature 409, 36-37.
Potyrailo R A (2011), "Bio-inspired device offers new model for vapor sensing", SPIE Newsroom, 10.1117/2.1201103.003568.
Russell P (2003), "Photonic crystal fibers", Science 299, 358-362.
Saranathan V, Osuji C O, Mochrie S G J, Noh H, Narayanan S, Sandy A, Dufresne E R & Prum R O (2010), "Structure, function, and self-assembly of single network gyroid (I4132) photonic crystals in butterfly wing scales", Proc. Natl Acad. Sci. USA 107, 11676-11681.
Schenk F (2009), "Nature's fluctuating colour captured on canvas?", Int. J. Design & Nature and Ecodynamics 4(3), 1-11.
Schenk F & Parker A (2011), "Iridescent color: from nature to the painter's palette", Leonardo 4(2) [no page numbers].
Shawkey M D, Morehouse N I & Vukusic, P (2009), "A protean palette: colour materials and mixing in birds and butterfiles", J. R. Soc. Interface 6, S221-S231.
Stavenga D G, Leertouwer H L, Marshall N J & Osorio D (2011), "Dramatic colour changes in a bird of paradise caused by uniquely structured breast feather barbules", Proc. R. Soc. B 278, 2098-2104.
Stefik M, Wang S, Hovden R, Sai H, Tate M W, Muller D A, Steiner U, Gruner S M & Wiesner U (2012), "Networked and chiral nanocomposites from ABC triblock terpolymer coassembly with transition metal oxide nanoparticles", J. Mater. Chem. 22, 1078-1087.
Tao A R, DeMartini D G, Izumi M, Sweeney A M, Holt A L & Morse D E (2010), "The role of protein assembly in dynamically tunable bio-optical tissues", Biomaterials 31, 793-801.
Turner M D, Schröder-Turk G E & Gu M (2011), "Fabrication and characterization of three-dimensional biomimetic chiral composites", Opt. Express 19(10), 10001-10008.
Vukusic P and Sambles J R (2003), "Photonic structures in biology", Nature 424, 852-855.
Vukusic P (2004), "Natural photonics", Physics World 17 (2), 35-39.
Trzeciak T M & Vukusic P (2009), "Photonic crystal fiber in the polychaete worm Pherusa sp.", Phys. Rev. E 80, 061908.
Wolpert H D (2009), "Optical filters in nature", Optics and Photonics News 20(2), 22-27.
05/30/12
[ TOP ]